Introduction
Beneath every productive agricultural field exists a complex, living ecosystem largely invisible to the naked eye but fundamental to crop productivity and environmental sustainability. The soil microbiome—comprising bacteria, fungi, archaea, protists, and viruses—represents one of the most diverse communities on Earth, with a single gram of soil potentially harboring billions of microorganisms and thousands of species. This microscopic community serves as the biological engine of soil fertility, influencing nutrient cycling, organic matter decomposition, soil structure, plant health, and crop productivity. In recent decades, advances in molecular biology, particularly next-generation sequencing and bioinformatics, have revolutionized our ability to characterize soil microbial communities and understand their functional contributions to agroecosystems. This enhanced understanding presents unprecedented opportunities to manage the soil microbiome as a tool for sustainable intensification of agriculture—producing more with fewer external inputs while enhancing ecosystem services. This article examines the scientific foundations of soil microbiome function, analyzes management approaches to enhance beneficial microbial communities, evaluates applications across different agricultural systems, addresses implementation challenges, presents case studies of successful microbiome management, and explores emerging directions in this rapidly evolving field.
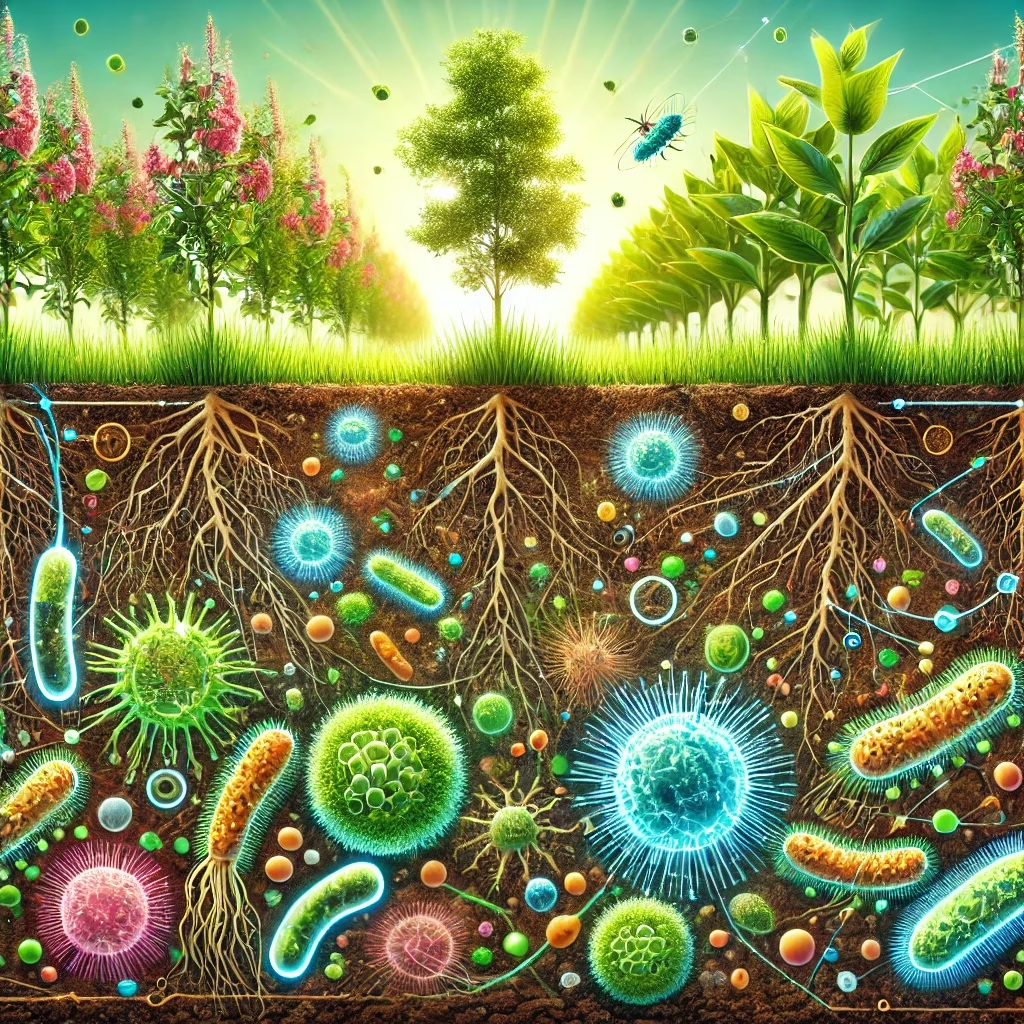
The Science of Soil Microbiome Function
Understanding soil microbiome contributions to agriculture requires examining multiple dimensions of microbial ecology and plant-microbe interactions:
- Composition and Diversity
Soil microbial communities exhibit remarkable taxonomic and functional diversity:- Bacterial communities: Typical agricultural soils contain 109-1010 bacterial cells per gram, representing 2,000-8,500 operational taxonomic units (OTUs). Research shows that productive agricultural soils typically host 15-40% greater bacterial diversity than degraded soils, with certain phyla like Proteobacteria, Actinobacteria, and Acidobacteria comprising 40-80% of bacterial communities.
- Fungal communities: Fungal abundance ranges from 105-106 cells per gram in agricultural soils, with 200-1,500 species typically present. Studies demonstrate that the ratio of fungal to bacterial biomass ranges from 0.3-1.2 in arable soils to 1.5-3.0 in pasturelands and forests, with higher ratios generally associated with reduced tillage and increased carbon sequestration.
- Microbial networks: Soil microbes form complex interaction networks through competitive, cooperative, and commensal relationships. Network analysis reveals that healthy agricultural soils typically contain 30-60% more microbial co-occurrence relationships than degraded soils, with key “hub” taxa often serving as central connectors that maintain community stability.
- Functional redundancy: Multiple microbial taxa often perform similar ecological functions, creating system resilience. Research demonstrates that high-functioning soils maintain 40-80% functional redundancy for critical processes like nitrogen fixation and phosphorus solubilization, providing stability under stress conditions.
- Plant-Microbe Interactions
Plants actively shape their microbial communities through multiple mechanisms:- Rhizodeposition: Plants release 20-40% of photosynthetically fixed carbon as root exudates, mucilage, and sloughed cells. Studies using isotope tracing show that these carbon inputs selectively enrich specific microbial taxa, with plants allocating 5-20% more carbon to beneficial microbes when under stress.
- Microbiome recruitment: Plants actively attract beneficial microorganisms through molecular signaling. Research demonstrates that crop varieties can differ by 15-35% in their ability to recruit beneficial microbes like nitrogen-fixing bacteria and mycorrhizal fungi, representing an untapped breeding target.
- Microbial services to plants: Microbes provide multiple benefits to host plants. Meta-analyses show that effective plant-microbe partnerships can provide 20-40% of plant nitrogen requirements, increase phosphorus acquisition by 30-50%, and reduce pathogen infection by 40-80% compared to plants with disrupted microbiomes.
- Induced systemic resistance: Beneficial microbes prime plant immune systems against pathogens. Studies demonstrate that plants with well-established beneficial microbiomes show 30-70% higher expression of defense-related genes when challenged by pathogens compared to sterile-grown plants.
- Nutrient Cycling and Soil Fertility
Microorganisms drive essential nutrient transformations in soil:- Nitrogen transformations: Microbial processes including fixation, mineralization, nitrification, and denitrification control soil nitrogen availability. Research indicates that biological nitrogen fixation can contribute 15-150 kg N/ha annually in agricultural systems depending on management, representing 10-50% of crop nitrogen requirements.
- Phosphorus solubilization: Microbes release organic acids and enzymes that convert unavailable phosphorus forms into plant-available nutrients. Studies show that phosphorus-solubilizing microorganisms can increase plant-available phosphorus by 20-40% in phosphorus-limited soils, potentially reducing fertilizer requirements by 15-30%.
- Carbon cycling: Microbial decomposition of organic matter influences both nutrient release and carbon sequestration. Research demonstrates that shifts in microbial community composition can alter decomposition rates by 15-50%, with fungal-dominated communities typically slowing decomposition and enhancing carbon storage.
- Micronutrient availability: Specialized microbes influence iron, zinc, and other micronutrient dynamics. Field studies show that beneficial siderophore-producing bacteria can increase micronutrient uptake by 10-25% in calcareous soils where these elements are often limiting.
- Ecological Resilience and Stress Protection
Diverse microbiomes enhance system stability under challenging conditions:- Drought resilience: Specialized microorganisms help plants withstand water limitation. Research shows that drought-adaptive microbiomes can maintain crop yields under 30-50% reduced irrigation by enhancing osmotic adjustment, modifying root architecture, and producing protective compounds.
- Disease suppression: Balanced microbial communities naturally inhibit pathogen proliferation. Studies in disease-suppressive soils demonstrate 50-95% reductions in root disease incidence compared to conducive soils through competition, antagonism, and induced plant resistance.
- Abiotic stress mitigation: Beneficial microbes produce stress-protective compounds. Experimental evidence shows that plants inoculated with stress-protective microbiomes maintain 15-40% higher photosynthetic efficiency under heat, cold, or salinity stress compared to non-inoculated controls.
- Recovery after disturbance: Microbiome composition influences ecosystem recovery rates. Research demonstrates that fields with greater microbial diversity recover key functions like nitrogen cycling and organic matter decomposition 30-60% faster following disturbances like flooding or extreme temperature events.
Management Approaches for Enhanced Soil Microbiome Function
Multiple agricultural practices can be implemented to cultivate beneficial soil microbiomes:
- Organic Matter Management
Carbon inputs fundamentally shape soil microbial communities:- Diverse carbon sources: Providing varied organic inputs supports diverse microbial communities. Research shows that combining different organic amendments (crop residues, manure, compost) increases microbial diversity indices by 20-40% compared to single-source amendments.
- Compost application: Mature compost introduces beneficial microorganisms and stable carbon compounds. Studies demonstrate that compost applications of 5-10 tons/ha annually increase microbial biomass by 30-70% and enzyme activities by 40-100% while enhancing nutrient cycling and disease suppression.
- Cover cropping: Growing cover crops during otherwise fallow periods provides continuous carbon flow to soil microbes. Meta-analyses indicate that diverse cover crop mixtures increase microbial biomass carbon by 15-40% and potentially mineralizable nitrogen by 10-25% compared to bare fallows.
- Residue management: Retaining and managing crop residues supports microbial continuity. Research shows that retaining at least 30% of crop residues increases fungal:bacterial ratios by 0.2-0.5 units and enhances aggregate stability by 15-30% through fungal hyphae networks.
- Reduced Soil Disturbance
Minimizing disruption preserves microbial habitats and networks:- Conservation tillage: Reducing tillage intensity protects soil structure and fungal networks. Studies demonstrate that no-till systems contain 60-120% higher mycorrhizal fungi abundance and 20-50% greater microbial biomass in surface soils compared to conventional tillage.
- Controlled traffic farming: Limiting compaction preserves soil pore networks that serve as microbial habitats. Research shows that controlled traffic systems maintain 15-25% higher soil biological activity and 20-40% better gas exchange, supporting aerobic microbial communities.
- Perennial integration: Including perennial crops in rotations provides stable microbial habitats. Studies demonstrate that perennial grain fields contain 40-70% higher fungal diversity and 25-45% more complex microbial interaction networks compared to annual grain fields.
- Strategic soil disturbance: Targeted, limited soil disturbance can provide specific benefits. Research indicates that strategic shallow tillage at appropriate intervals can reduce soil-borne pathogen loads by 30-50% while maintaining 70-85% of the beneficial microbial community structure present in undisturbed systems.
- Microbial Inoculation
Directly introducing beneficial microorganisms supplements indigenous communities:- Rhizobial inoculants: Applying nitrogen-fixing bacteria to legume crops ensures effective symbiosis. Field trials consistently show 15-80% yield increases in newly established legume crops following proper inoculation, with economic returns of $5-20 per dollar invested in inoculant.
- Mycorrhizal fungi applications: Introducing arbuscular mycorrhizal fungi enhances nutrient acquisition networks. Research demonstrates that appropriate mycorrhizal inoculation increases phosphorus uptake by 20-50% in phosphorus-limited soils and improves water acquisition during drought by 15-30%.
- Plant growth-promoting rhizobacteria: Applying beneficial bacterial strains enhances multiple plant functions. Meta-analyses show average yield increases of 5-30% following PGPR inoculation, with effects varying by crop, soil type, and prevailing environmental stressors.
- Complex microbial consortia: Multi-species inoculants provide complementary functions. Studies indicate that well-designed microbial consortia outperform single-strain inoculants by 15-40% in field conditions by providing multiple simultaneous benefits and enhancing establishment success.
- Chemical Input Management
Carefully managing agrochemicals protects microbiome integrity:- Reduced fungicide use: Minimizing fungicide applications preserves beneficial fungi. Research shows that reducing fungicide use by 30-50% through precision application and integrated management maintains 90-95% disease control while preserving 20-40% greater mycorrhizal colonization.
- Selective pesticides: Choosing compounds with lower impacts on non-target microbes. Comparative studies demonstrate that selective pesticides preserve 30-70% more beneficial microbial diversity than broad-spectrum alternatives while maintaining equivalent pest control.
- Fertilizer optimization: Balancing nutrient additions to avoid microbial disruption. Studies show that reducing nitrogen fertilizer by 15-30% below conventional rates while using enhanced efficiency formulations maintains crop yields while increasing nitrogen-fixing bacterial populations by 20-50%.
- Integrated management: Combining biological and chemical approaches in balanced systems. Research demonstrates that integrated systems using 40-60% lower chemical inputs supplemented with biological products maintain equivalent yields while supporting 25-45% higher microbial diversity.
- Agricultural System Design
Broader management systems influence microbiome development:- Crop diversity: Increasing rotational and spatial crop diversity enhances microbial diversity. Studies show that each additional crop in a rotation increases microbial diversity indices by 5-15% and functional gene abundance by 7-18%, with diminishing but positive returns as complexity increases.
- Livestock integration: Incorporating animals into cropping systems enhances microbiome development. Research demonstrates that integrated crop-livestock systems contain 20-40% higher microbial biomass and 15-30% greater enzymatic activities compared to specialized cropping systems, primarily due to diverse organic inputs.
- Agroforestry integration: Including trees within agricultural landscapes supports fungal networks. Studies show that agroforestry systems contain 50-100% higher ectomycorrhizal fungal diversity and 30-70% greater soil fungal:bacterial ratios compared to treeless systems in similar environments.
- Field margin management: Maintaining diverse non-crop areas provides microbial reservoirs. Research indicates that fields with diverse perennial borders show 10-25% higher recolonization rates of beneficial microbes following disturbance compared to fields without such margins.
Applications and Benefits Across Agricultural Systems
Microbiome management offers multiple benefits that vary across different agricultural contexts:
- Annual Cropping Systems
Row crop and grain production systems benefit from enhanced microbiomes:- Fertilizer use efficiency: Optimized microbiomes improve nutrient capture and cycling. Field trials demonstrate that crops with well-managed microbiomes require 15-30% less applied nitrogen and phosphorus to achieve equivalent yields, particularly when biological nitrogen fixation and phosphorus solubilization are enhanced.
- Soil structure improvement: Microbial production of binding agents enhances aggregation. Research shows that promoting fungal-bacterial balance through organic matter management increases water-stable aggregates by 20-45% and reduces erosion potential by 30-60% compared to microbiologically degraded soils.
- Yield stability: Diverse microbiomes buffer against stress conditions. Long-term studies indicate that fields with managed microbiomes show 15-30% less yield variability across seasons, particularly during drought years when yields can be 20-40% higher than in fields with degraded microbiomes.
- Disease management: Suppressive soils reduce pathogen impacts without fungicides. Research demonstrates that fields with managed microbiomes experience 40-70% lower incidence of soilborne diseases like Fusarium wilt, Rhizoctonia root rot, and Pythium damping-off compared to fields with simplified microbiomes.
- Horticultural Production Systems
Vegetable, fruit, and specialty crop production offers unique microbiome applications:- Replant disease mitigation: Microbiome management addresses mono-culture challenges. Studies in orchard systems show that establishing diverse cover crops and applying compost reduces replant disease incidence by 50-80% compared to fumigation alone, while supporting 30-50% greater tree growth in the establishment years.
- Quality enhancement: Beneficial microbes influence crop quality parameters. Research demonstrates that fruits and vegetables grown with optimized root microbiomes show 10-30% higher antioxidant content, 5-15% increased sugar levels, and 10-20% improved shelf life compared to conventionally grown crops.
- Water use efficiency: Microbiome-mediated improvements in root function enhance water acquisition. Field trials in vegetable production demonstrate that crops with mycorrhizal-rich soils maintain productivity with 15-30% less irrigation, particularly under deficit irrigation regimes.
- Controlled environment integration: Even indoor and greenhouse production benefits from microbiome management. Studies show that introducing complex microbiomes to soilless media increases yield stability by 10-25% and reduces disease incidence by 30-60% compared to sterile growing systems.
- Permanent Crop Systems
Orchards, vineyards, and other perennial crops present long-term microbiome opportunities:- Establishment success: Early microbiome development enhances perennial crop establishment. Research demonstrates that trees and vines receiving beneficial microbiome inoculations and establishment care show 20-40% greater growth rates and 30-50% lower mortality during the first three years.
- Drought resilience: Well-developed mycorrhizal networks improve water acquisition. Studies in vineyards and orchards show that established mycorrhizal networks enable 15-35% greater production under water limitation compared to newly planted or fumigated systems lacking fungal networks.
- Soil carbon sequestration: Long-term perennial systems with managed microbiomes accumulate carbon. Research indicates that orchards and vineyards with cover crops and minimal soil disturbance sequester 0.5-2.0 tons of carbon per hectare annually, with microbial transformation of plant inputs accounting for 40-60% of this sequestration.
- Terroir and crop quality: Microbiomes influence distinctive product characteristics. Studies in wine grape production demonstrate that vineyard-specific microbiomes influence 10-30% of the metabolite profile in grapes that contributes to wine sensory properties and regional distinctiveness.
- Rangeland and Pasture Systems
Grazing lands benefit from specialized microbiome management:- Forage productivity: Enhanced soil microbiomes increase grass and legume production. Research shows that pastures with diverse microbial communities produce 15-40% more biomass and contain 10-25% higher crude protein than degraded pastures, particularly during stress periods.
- Legume establishment and persistence: Effective rhizobial partnerships improve nitrogen fixation. Field trials demonstrate that proper rhizobial inoculation increases clover and alfalfa establishment by 30-80% and persistence by 1-3 years in mixed pastures while fixing 50-200 kg N/ha annually.
- Carbon sequestration: Grazing management influences microbial carbon processing. Studies show that adaptive multi-paddock grazing supporting diverse microbiomes increases soil carbon accumulation by 0.5-3.0 tons per hectare annually compared to continuous grazing, with fungal-bacterial ratios serving as key indicators of carbon storage potential.
- Invasive species resistance: Intact soil microbiomes enhance native plant competitiveness. Research demonstrates that rangelands with well-functioning microbiomes show 20-50% greater resistance to invasive weed establishment through microbial feedback mechanisms that preferentially benefit native species.
- Degraded Land Restoration
Microbiome reconstruction plays a critical role in rehabilitating damaged landscapes:- Accelerated succession: Inoculation with appropriate microbiomes speeds recovery processes. Studies show that severely degraded lands receiving native soil inoculum along with appropriate plant materials recover key ecosystem functions 3-10 times faster than non-inoculated areas.
- Heavy metal and pollutant remediation: Specialized microbes transform or immobilize contaminants. Research demonstrates that bioaugmentation with metal-tolerant microorganisms can reduce bioavailable heavy metals by 30-70% while supporting 40-90% greater plant establishment on contaminated sites.
- Saline soil reclamation: Salt-tolerant microbiomes facilitate cropping on saline soils. Field trials show that halophilic bacterial inoculants combined with organic amendments reduce soil electrical conductivity by 15-40% over 2-3 years while enabling 30-60% greater productivity of salt-tolerant crops.
- Post-fire recovery: Microbiome restoration accelerates ecosystem recovery after fires. Research indicates that areas receiving soil microbiome transfers from unburned sites show 40-70% faster vegetation recovery and 50-80% lower erosion rates compared to sites recovering through natural processes alone.
Challenges and Implementation Considerations
Despite promising potential, several challenges must be addressed for effective microbiome management:
- Ecological Complexity and Predictability
The inherent complexity of soil microbiomes creates practical challenges:- Context dependency: Microbial functions vary with environmental conditions. Meta-analyses reveal that identical microbiome management practices produce highly variable results (coefficient of variation 30-70%) across different soil types, climates, and cropping systems, requiring localized adaptation rather than universal protocols.
- Temporal dynamics: Microbiomes change seasonally and successionally. Research shows that beneficial inoculants typically decline by 60-90% within 30-60 days after application unless supported by appropriate management, highlighting the need for establishment strategies rather than one-time interventions.
- Priority effects and biological legacies: Existing microbiomes influence the success of interventions. Studies demonstrate that heavily disturbed soils with degraded microbiomes often require 3-5 years of consistent management to rebuild beneficial communities, while soils with some biological legacy recover 3-10 times faster.
- Functional redundancy challenges: Multiple organisms perform similar functions but respond differently to management. Research indicates that functional gene abundance often predicts microbial services more accurately than taxonomic composition, suggesting management should target functions rather than specific taxa.
- Technical and Practical Barriers
Implementation faces several practical hurdles:- Inoculant quality issues: Commercial microbial products vary greatly in quality and efficacy. Market analyses show that 20-40% of commercial inoculants contain non-viable microorganisms or contaminants, with product efficacy varying by 0-80% depending on manufacturing quality, formulation, and application method.
- Delivery and establishment challenges: Getting microbes to their target location and ensuring establishment is difficult. Research demonstrates that innovative delivery systems like seed coatings, biofilms, and encapsulation technologies improve microbial survival by 30-90% and establishment by 20-60% compared to traditional liquid applications.
- Shelf life limitations: Many beneficial microbes lose viability during storage. Studies show that most non-spore-forming bacteria lose 50-90% viability within 2-6 months under ambient conditions, necessitating cold storage, stabilizing formulations, or on-farm production systems.
- Equipment and infrastructure needs: Some practices require specialized equipment or infrastructure. Economic analyses indicate that initial investments of $5,000-50,000 for compost production facilities or cover crop management equipment may be necessary, creating adoption barriers despite positive returns on investment of 20-60% over 3-5 years.
- Economic and Knowledge Constraints
Financial and informational barriers affect implementation:- Upfront costs vs. long-term benefits: Many microbiome management practices incur immediate costs while benefits accrue over years. Economic modeling shows that transition periods of 2-5 years are common before positive returns emerge, creating cash flow challenges despite internal rates of return of 15-40% over 10-year periods.
- Risk perception: Uncertainty about outcomes affects adoption decisions. Surveys indicate that farmers perceive 20-50% greater risk in microbiome management approaches compared to conventional chemical-intensive practices, even when objective analysis shows equivalent or lower actual risk.
- Knowledge intensity: Effective microbiome management requires ecological understanding. Research shows that successful practitioners typically have access to 3-5 times more information resources and participate in 2-3 times more knowledge-sharing networks than non-adopters.
- Monitoring challenges: Assessing microbiome status remains difficult and expensive. Current soil health tests capturing microbiome functions cost $20-200 per sample, while comprehensive microbiome analysis costs $300-1,000 per sample, limiting feedback for adaptive management.
- Regulatory and Policy Environment
External factors influence adoption feasibility:- Microbial product regulation: Approval processes for microbial products vary widely by jurisdiction. Regulatory analyses show that bringing new microbial products to market costs $1-5 million and takes 3-8 years in regions with strict regulatory frameworks compared to $50,000-500,000 and 1-3 years in regions with streamlined approaches.
- Incentive program alignment: Agricultural subsidies and incentives often don’t recognize microbiome management. Policy reviews demonstrate that realigning $15-50/hectare of existing agricultural supports toward soil health practices increases adoption rates by 30-80% without requiring additional public expenditure.
- Land tenure effects: Insecure land tenure discourages long-term microbiome investment. Studies show that farmers with annual leases implement 60-80% fewer microbiome-building practices than owner-operators, while leases exceeding 5 years show only 10-20% implementation gaps.
- Certification systems: Marketplace recognition for microbiome management remains limited. Market analyses indicate that recognized certification for “soil health” or “microbiome-managed” products could generate 5-15% price premiums, potentially offsetting 30-70% of additional management costs.
Case Studies of Successful Implementation
Examining specific success stories provides insights into effective implementation approaches:
- Disease-Suppressive Soils in Dutch Flower Bulb Production
The Netherlands’ flower bulb industry has developed practical microbiome management for disease control:- Challenge addressed: Pythium root rot and Fusarium basal rot previously required methyl bromide fumigation, which has been phased out globally due to environmental concerns.
- Microbiome approach: Producers implemented a system combining compost additions (15-20 tons/ha annually), strategic cover cropping with specific grass-clover mixtures, and controlled application of chitin-rich amendments that stimulate chitinolytic bacteria antagonistic to fungal pathogens.
- Results achieved: Fields under microbiome management for 3+ years show 60-90% lower disease incidence than conventionally managed fields. Producers have reduced fungicide applications by 50-70% while maintaining or increasing yield and quality. Economic analysis shows 15-25% higher net returns despite initial 3-year transition costs.
- Key success factors: Long-term research collaboration between growers and scientists, development of simple field indicators for suppressive potential, and formation of grower learning networks to share experiences accelerated adoption beyond initial demonstration sites.
- Dryland Wheat Production in the Pacific Northwest (USA)
Farmers in low-rainfall wheat production regions have leveraged microbiome management for resilience:- Challenge addressed: Dryland wheat production faces water limitation, soil erosion, and declining soil organic matter, with yields historically plateauing despite technological advances.
- Microbiome approach: Producers implemented reduced tillage systems with diverse crop rotations (expanding from wheat-fallow to 3-4 crop sequences), maintained high residue cover, and introduced mycorrhizal-supporting cover crop mixes during traditional fallow periods.
- Results achieved: After 5-7 years, participating farms documented 40-80% higher soil fungal diversity, 30-45% greater water infiltration rates, and 15-30% improved water use efficiency. Wheat yields increased by 7-15% while requiring 20-30% less nitrogen fertilizer. Yield stability during drought years improved by 30-50% compared to conventional systems.
- Key success factors: Regional research stations established long-term demonstration sites, early adopters received risk mitigation support during transition, and quantification of water conservation benefits provided tangible evidence of value in water-limited environments.
- Brazilian Sugarcane and Plant Growth-Promoting Bacteria
Brazil’s sugarcane industry has successfully scaled microbial inoculation:- Challenge addressed: Nitrogen fertilizer represents 40% of production costs and creates significant environmental impacts in sugarcane production, while yields faced plateaus in conventional systems.
- Microbiome approach: Researchers identified associative nitrogen-fixing bacteria (primarily Gluconacetobacter diazotrophicus, Herbaspirillum seropedicae, and Azospirillum amazonense) naturally occurring in sugarcane. These were developed into commercial inoculants and integrated into planting systems.
- Results achieved: Inoculated sugarcane reduces nitrogen fertilizer requirements by 30-50% while maintaining equivalent yields. Some operations report 5-15% yield increases beyond conventional practices when inoculation is combined with optimized management. The approach has been adopted on over 3 million hectares, saving approximately 150 million kg of nitrogen annually.
- Key success factors: Public-private research partnerships ensured effective technology transfer, quality control systems for inoculant production maintained efficacy at scale, and clear economic benefits drove rapid industry adoption once the technology was proven.
- California Almond Orchard Soil Health Initiative
Almond producers have increasingly adopted microbiome management in this high-value perennial system:- Challenge addressed: Intensive almond production traditionally involved clean cultivation, fumigation before planting, high synthetic input dependency, and declining soil function over orchard lifespan.
- Microbiome approach: Progressive producers implemented whole orchard recycling of removed trees, established diverse floor vegetation, applied compost (5-10 tons/ha biennially), reduced soil disturbance, and inoculated with mycorrhizal fungi during planting.
- Results achieved: Orchards under microbiome management for 4+ years demonstrate 20-35% higher soil organic matter, 30-60% greater microbial biomass, and 25-40% increased water infiltration rates. Young trees establish 30-50% faster after whole orchard recycling, while mature orchards require 15-25% less irrigation while maintaining yields. Production costs typically decrease by 10-20% primarily through reduced irrigation and fertility inputs.
- Key success factors: Industry organization support provided research funding and knowledge dissemination, cost-share programs helped offset transition costs, and quantification of water savings created compelling economic justification in water-limited regions.
- East African Smallholder Rhizobium Networks
Small-scale farmers have benefited from legume inoculation programs:- Challenge addressed: Bean and soybean production in East Africa was limited by poor nodulation, with yields averaging only 20-30% of potential due to inadequate nitrogen fixation in degraded soils lacking appropriate rhizobia.
- Microbiome approach: A network of country-based inoculant production facilities was established, along with farmer training programs and supply chain development. Context-specific rhizobial strains were selected for local conditions rather than using generic commercial products.
- Results achieved: Properly inoculated legume crops show 50-120% yield increases compared to non-inoculated crops in first-time fields. Biological nitrogen fixation increased from 10-30 kg N/ha to 50-120 kg N/ha, improving subsequent cereal crop yields by 30-60% without additional inputs. The program has reached over 1.5 million smallholder farmers with benefit-cost ratios exceeding 10:1.
- Key success factors: Local production reduced costs to 1/5 of imported inoculants, quality control systems ensured product efficacy, and intensive farmer education created understanding of the invisible microbial processes driving benefits.
Future Directions and Emerging Approaches
Several frontiers promise to further enhance soil microbiome management capabilities:
- Advanced Monitoring and Diagnostic Tools
Improved detection and analysis methods will enable more precise management:- Field-deployable sequencing: Portable DNA sequencing technologies enable on-farm microbiome analysis. Early implementations show 70-90% concordance with laboratory methods at 10-30% of the cost and time, enabling real-time management decisions based on microbiome status.
- Functional gene quantification: Measuring key functional genes rather than taxonomic composition provides direct insight into microbial services. Research demonstrates that quantifying genes involved in nitrogen fixation (nifH), phosphorus solubilization (phoD), and disease suppression (2,4-DAPG) predicts agricultural outcomes 30-50% more accurately than species-level analyses.
- Sensor networks and automated monitoring: Continuous measurement of microbial activity indicators allows responsive management. Early field trials of soil respiration sensor networks show 20-40% improvement in management timing decisions compared to periodic manual assessments.
- Machine learning integration: Advanced analytics extract meaningful patterns from complex microbiome data. Models combining soil microbiome data with environmental parameters demonstrate 25-50% improved prediction accuracy for crop productivity and pest suppression compared to conventional models.
- Designer Microbiomes and Synthetic Communities
Intentionally constructed microbial consortia offer improved performance:- Minimal effective communities: Identifying the smallest functional groups of microorganisms that deliver specific services. Research shows that carefully designed 5-12 species consortia can provide 70-90% of the benefits observed in diverse natural communities of hundreds of species when targeting specific functions like nitrogen fixation or pathogen suppression.
- Complementary trait selection: Assembling microbes with non-overlapping ecological functions enhances overall service delivery. Studies demonstrate that consortia composed of members with complementary carbon metabolism, spatial niche occupation, and temporal activity patterns outperform random assemblages by 30-70% in colonization success and functional stability.
- Microbe-microbe compatibility: Engineering microbial teams with positive interactions improves performance. Laboratory and field research shows that screening for compatible microorganisms before formulation increases consortium efficacy by 40-80% compared to combining individually effective strains without compatibility testing.
- Phage inclusion: Incorporating bacteriophages helps control specific pathogen populations. Early field trials demonstrate that adding target-specific phages to microbial consortia enhances disease suppression by 20-50% through precise control of bacterial pathogens while preserving beneficial community members.
- Plant-Microbiome Engineering
Optimizing plant-microbial partnerships through breeding and biotechnology:- Root exudate modification: Selecting plants that release specific compounds attracting beneficial microbes. Research shows that maize lines bred for altered benzoxazinoid profiles recruit 30-60% more protective Pseudomonas bacteria, resulting in 40-70% lower root herbivory damage.
- Symbiosis genes: Enhancing plants’ ability to form beneficial partnerships. Experimental work demonstrates that optimizing legume-rhizobia symbiosis genes can increase nitrogen fixation by 15-40%, while transferring mycorrhizal reception pathways to currently non-mycorrhizal crops shows promise for expanding these partnerships.
- Microbiome-aware breeding: Selecting crop varieties based on microbiome interaction capacity. Field trials indicate that incorporating microbiome recruitment traits into breeding programs increases yield stability by 10-30% across diverse environments compared to conventional breeding focused solely on direct plant traits.
- Endophyte engineering: Modifying internal plant-dwelling microbes for enhanced function. Research with engineered bacterial endophytes shows potential for improving nitrogen use efficiency by 20-35% and drought tolerance by 15-40% through production of specific hormones and osmoprotectants within plant tissues.
- Integrated Digital-Biological Systems
Combining technology with biological insights enhances precision and efficacy:- Digital decision support: Software tools integrating soil, climate, and biological data guide microbiome management decisions. Early adopters report 15-30% better outcomes and 20-40% reduced input costs using microbiome-aware decision support systems compared to standard agricultural recommendations.
- Precision biologicals application: Site-specific delivery of biological inputs based on soil and crop needs. Field-scale trials demonstrate that variable-rate bioinoculant application matched to soil organic matter and texture maps improves establishment success by 30-60% while reducing product use by 20-45%.
- Autonomous microbiome monitoring: Automated sampling and analysis systems provide continuous feedback. Prototype robotic systems for soil microbiome assessment reduce monitoring costs by 60-80% while increasing sampling frequency by 5-10 times compared to manual methods.
- Predictive microbiome modeling: Simulating microbiome responses to management before field implementation. Validation studies show that advanced microbiome models incorporating weather forecasts, soil conditions, and management history predict functional responses with 60-75% accuracy, enabling scenario testing before implementation.
- Landscape and Policy-Level Approaches
Broader frameworks to support microbiome management adoption:- Watershed microbiome management: Coordinating practices across landscapes enhances outcomes. Research demonstrates that synchronized microbiome-enhancing practices across 50-60% of a watershed can reduce nutrient runoff by 30-70% and improve downstream water quality 2-4 times more effectively than disconnected individual farm implementations.
- Ecosystem service marketplaces: Monetary recognition of microbiome-related environmental benefits. Emerging carbon and water quality markets valuing soil health improvements at $30-150/hectare annually provide financial incentives representing 30-100% of implementation costs for microbiome-enhancing practices.
- Microbiome-aware policy frameworks: Government programs that recognize and reward microbial contributions to agricultural resilience. Policy analyses indicate that restructuring 15-25% of existing agricultural subsidies toward verified soil health outcomes could trigger 3-5 fold increases in microbiome-enhancing practice adoption.
- Regional microbiome resource centers: Infrastructure supporting local adaptation and implementation. Case studies of regional centers providing microbiome analytics, microbial resources, and technical support show 40-70% higher successful implementation rates compared to areas without such support structures.
Conclusion
The soil microbiome represents an untapped resource for enhancing agricultural sustainability, productivity, and resilience. The complex communities of bacteria, fungi, and other microorganisms living beneath our feet perform essential functions in nutrient cycling, organic matter management, plant health protection, and abiotic stress mitigation. As our understanding of these communities advances, so does our ability to manage them for agricultural benefit rather than inadvertently disrupting their functions through intensive practices.
The evidence demonstrates that well-managed soil microbiomes contribute significantly to agricultural performance across diverse metrics. They can provide 10-50% of crop nitrogen requirements through biological fixation, increase phosphorus availability by 20-40%, reduce disease incidence by 40-80%, enhance water use efficiency by 15-30%, and improve yield stability by 15-40% during stressful conditions. These benefits translate to reduced input costs, increased resilience to climate variability, and enhanced environmental outcomes including carbon sequestration and reduced nutrient leaching.
Multiple management approaches can enhance soil microbiome function, including organic matter additions, reduced soil disturbance, crop diversification, strategic inoculation, and careful input management. The most successful implementations typically combine multiple practices into coherent systems adapted to local conditions rather than relying on single interventions. Despite implementation challenges related to knowledge requirements, transition costs, and technological limitations, the growing body of successful case studies across diverse agricultural systems demonstrates that practical, economically viable microbiome management is increasingly feasible.
Looking forward, emerging technologies in microbiome analysis, synthetic community development, plant-microbe engineering, and digital integration promise to further enhance our ability to work with soil microbial communities rather than against them. As agriculture faces mounting challenges from climate change, resource limitations, and environmental concerns, the soil microbiome offers a biological pathway to address multiple constraints simultaneously while reducing dependency on external inputs and enhancing ecosystem services.
The transition to microbiome-informed agriculture requires shifts in research priorities, education, policy frameworks, and market systems—but the potential rewards justify these investments. By recognizing soil not merely as a growing medium but as a living ecosystem whose microbial components actively contribute to agricultural productivity, we can harness billions of microscopic allies in creating more sustainable and resilient food production systems for future generations.
References
- Fierer, N. (2017). Embracing the unknown: Disentangling the complexities of the soil microbiome. Nature Reviews Microbiology, 15(10), 579-590.
- Bender, S. F., Wagg, C., & van der Heijden, M. G. (2016). An underground revolution: Biodiversity and soil ecological engineering for agricultural sustainability. Trends in Ecology & Evolution, 31(6), 440-452.
- French, K. E. (2019). Harnessing synthetic biology for sustainable development. Nature Sustainability, 2(4), 250-252.
- Trivedi, P., Leach, J. E., Tringe, S. G., Sa, T., & Singh, B. K. (2020). Plant–microbiome interactions: From community assembly to plant health. Nature Reviews Microbiology, 18(11), 607-621.
- Chaparro, J. M., Sheflin, A. M., Manter, D. K., & Vivanco, J. M. (2012). Manipulating the soil microbiome to increase soil health and plant fertility. Biology and Fertility of Soils, 48(5), 489-499.
- Naylor, D., & Coleman-Derr, D. (2018). Drought stress and root-associated bacterial communities. Frontiers in Plant Science, 8, 2223.
- Banerjee, S., Schlaeppi, K., & van der Heijden, M. G. (2018). Keystone taxa as drivers of microbiome structure and functioning. Nature Reviews Microbiology, 16(9), 567-576.
- Wubs, E. R. J., van der Putten, W. H., Bosch, M., & Bezemer, T. M. (2016). Soil inoculation steers restoration of terrestrial ecosystems. Nature Plants, 2(8), 16107.
- Schmidt, R., Gravuer, K., Bossange, A. V., Mitchell, J., & Scow, K. (2018). Long-term use of cover crops and no-till shift soil microbial community life strategies in agricultural soil. PLoS One, 13(2), e0192953.
- Saad, M. M., Ghosh, S., & Verma, J. P. (2021). Metagenomics-guided mining of soil microbiome for novel nanobiomaterials to degrade agrochemicals. Environmental Research, 194, 110708.
- Lehmann, J., Bossio, D. A., Kögel-Knabner, I., & Rillig, M. C. (2020). The concept and future prospects of soil health. Nature Reviews Earth & Environment, 1(10), 544-553.
- Dubey, A., Malla, M. A., Khan, F., Chowdhary, K., Yadav, S., Kumar, A., Sharma, S., Khare, P. K., & Khan, M. L. (2019). Soil microbiome: A key player for conservation of soil health under changing climate. Biodiversity and Conservation, 28(8), 2405-2429.
- Schlatter, D., Kinkel, L., Thomashow, L., Weller, D., & Paulitz, T. (2017). Disease suppressive soils: New insights from the soil microbiome. Phytopathology, 107(11), 1284-1297.
- Sessitsch, A., Pfaffenbichler, N., & Mitter, B. (2019). Microbiome applications from lab to field: Facing complexity. Trends in Plant Science, 24(3), 194-198.
- Xie, J., Hu, L., Tang, J., Wu, X., Li, N., Yuan, Y., Yang, H., Zhang, J., Luo, S., & Chen, X. (2011). Ecological mechanisms underlying the sustainability of the agricultural heritage rice-fish coculture system. Proceedings of the National Academy of Sciences, 108(50), E1381-E1387.
If you want to learn more about Soil Microbiome Management, check out Agri AI : Smart Farming Advisor and feel free to ask any questions!
Leave a Reply