Introduction
The domestication and improvement of crop plants represents one of humanity’s greatest collaborative enterprises, spanning millennia and transforming wild species into the productive varieties that sustain global food systems. Plant breeding—the deliberate selection and improvement of plant populations for desired traits—has continuously evolved from early farmers’ unconscious selection to today’s sophisticated molecular approaches. The 21st century presents unprecedented challenges to agriculture, including climate change, population growth, evolving pest pressures, and resource constraints. Meeting these challenges requires breeding programs that develop crops with enhanced yield potential, nutritional quality, resource-use efficiency, and resilience to biotic and abiotic stresses. This article examines the evolution of plant breeding methodologies, analyzes the scientific principles underlying traditional and modern approaches, evaluates impacts and limitations of different breeding paradigms, addresses critical regulatory and access considerations, and explores how integrating diverse breeding strategies can enhance global food security and agricultural sustainability.
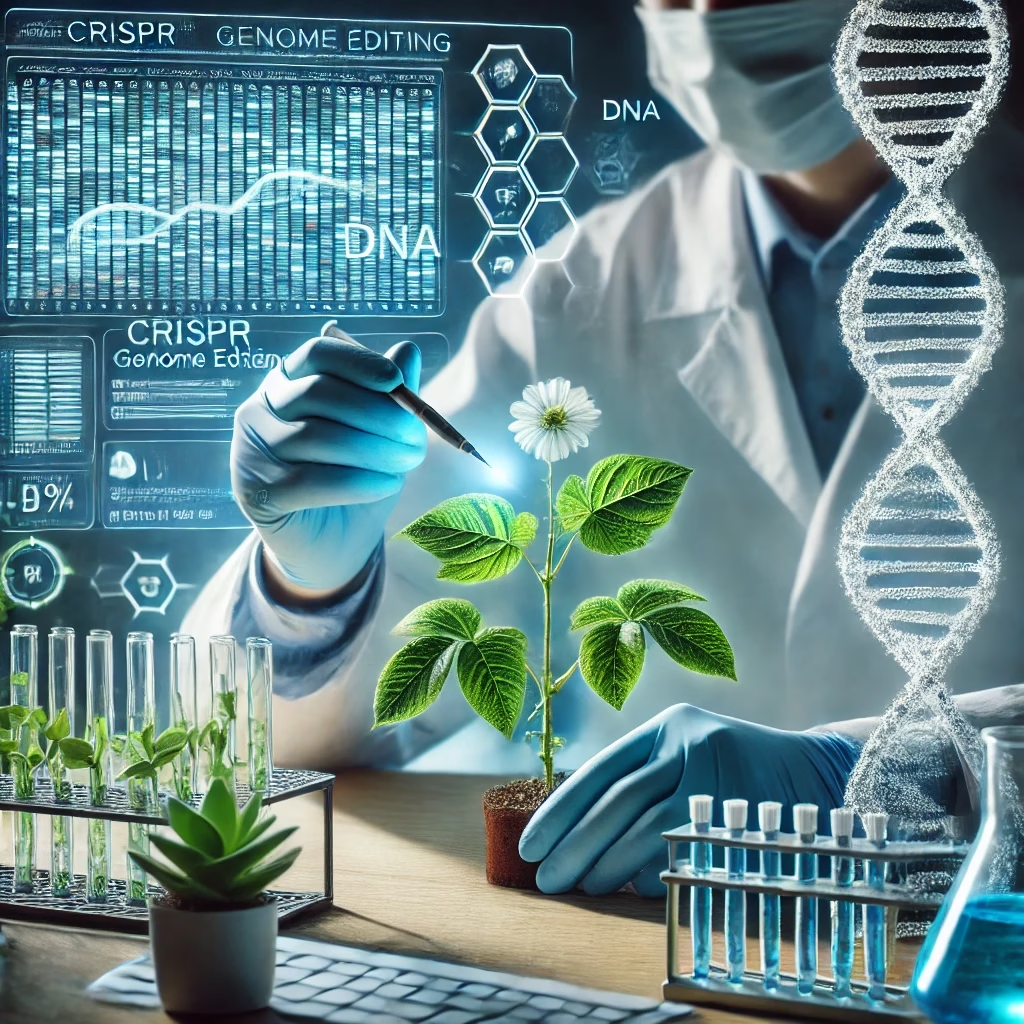
The Scientific Foundations of Plant Breeding
Plant breeding operates on several biological principles and processes that determine both its possibilities and limitations:
- Genetic Variation and Heritability
The fundamental prerequisite for breeding progress is genetic diversity that can be inherited:- Sources of variation: Natural genetic diversity arises from mutations, recombination, gene flow, and evolutionary processes. Research demonstrates that most crops retain only 10-20% of the genetic diversity present in their wild relatives, with modern elite varieties often possessing less than 30% of the diversity found in landraces. Studies in wheat show that breeding programs accessing novel germplasm sources improve genetic gain rates by 20-35% compared to programs recycling elite materials.
- Heritability components: Breeding progress depends on trait heritability—the proportion of phenotypic variation attributable to genetic factors. Meta-analyses show average broad-sense heritability of 0.4-0.7 for yield traits and 0.6-0.9 for quality traits across major crops. Narrow-sense heritability (additive genetic variance) typically ranges from 0.2-0.5 for complex traits and 0.5-0.8 for simpler traits, directly influencing selection response.
- Genetic architecture: Traits vary from simple Mendelian inheritance to complex polygenic control. Research using genome-wide association studies reveals that most agronomically important traits are controlled by 10-100+ genes, with effect sizes following exponential distributions where few loci explain 30-50% of genetic variance while many loci contribute small effects.
- Selection Theory and Response
Selection effectiveness depends on multiple interrelated factors:- Selection differential and intensity: The difference between selected individuals and the population mean, standardized by phenotypic standard deviation, determines potential gain. Studies show that commercial breeding programs typically apply selection intensities of 1-2.5%, retaining only the top-performing individuals from large populations.
- Selection accuracy: The correlation between selection criteria and true breeding value significantly impacts genetic gain. Research demonstrates that genomic selection has increased selection accuracy by 20-40% for complex traits compared to phenotypic selection alone, particularly for traits with low heritability.
- Genetic gain equation: The breeder’s equation (R = h² × S) predicts response to selection (R) based on narrow-sense heritability (h²) and selection differential (S). Long-term studies in maize show that annual genetic gains of 1-2% for yield have been maintained for decades through continuous selection, with accelerated rates of 2-3% in programs combining genomic and high-throughput phenotyping approaches.
- Reproductive Biology and Breeding Systems
Plant reproductive characteristics determine appropriate breeding strategies:- Mating systems: Self-pollinating crops (wheat, rice, tomato) contrast with cross-pollinating species (maize, alfalfa, brassicas) in population structure and breeding approaches. Research shows that hybrid breeding in traditionally self-pollinating crops can increase yields by 10-30% by capturing heterosis that remained unexploited in conventional breeding.
- Heterosis and inbreeding: Hybrid vigor (heterosis) results from the combination of divergent genetic backgrounds, while inbreeding typically reduces performance. Studies in maize demonstrate mid-parent heterosis of 15-45% for grain yield, with genetic distance between parents explaining 30-50% of heterosis magnitude when parents come from different heterotic groups.
- Reproductive barriers: Incompatibilities between species limit gene flow but can sometimes be overcome. Research shows that up to 30% of crop varieties contain introgressions from wild relatives or related species, contributing disease resistance, stress tolerance, and quality traits unobtainable from within primary gene pools.
- Genotype-Environment Interactions
Plant performance results from complex interactions between genetics and environment:- Performance stability: Genotypes vary in their response to environmental variation. Multi-environment trials show that genotype-environment interactions typically account for 20-40% of total variation in yield, necessitating extensive testing networks for variety development.
- Adaptation specificity: Varieties may excel in specific environments but perform poorly elsewhere. Research demonstrates that locally adapted varieties typically outperform broadly adapted materials by 10-25% under stress conditions, while broadly adapted varieties may yield 5-15% more across diverse favorable environments.
- Predictive modeling: Environmental factors can be incorporated into breeding decisions through various modeling approaches. Studies show that including environmental covariates in genomic prediction models increases prediction accuracy by 10-30% for stress-adaptive traits compared to models considering only genetic information.
Evolution of Plant Breeding Methodologies
Plant breeding has progressed through several methodological paradigms, each building upon previous approaches while introducing new capabilities:
- Mass Selection and Early Methodical Breeding
The foundation of plant improvement began with simple but effective approaches:- Farmer-led selection: For millennia, farmers selected superior individuals from genetically variable populations, gradually improving adaptation and performance. Archaeological evidence shows that this approach increased grain size in wheat by 25-40% within the first 2,000 years of cultivation.
- Landrace development: Local adaptation and farmer selection created thousands of genetically diverse but locally optimized varieties. Research demonstrates that landraces contain 3-5 times greater genetic diversity than modern varieties while providing adaptation to specific microclimates and farming systems.
- Early scientific selection: Organized selection methods emerged in the 18th-19th centuries, applying more systematic approaches. Historical analyses show that wheat yields in Europe increased 0.4-0.8% annually during the 19th century primarily through methodical mass selection and landrace improvement.
- Hybridization and Pedigree Breeding
Controlled crosses enabled more directed improvement:- Deliberate hybridization: Crossing complementary parents to create new genetic combinations became standard practice in the late 19th and early 20th centuries. Historical records demonstrate that wheat varieties derived from deliberate crosses showed 15-30% yield advantages over direct selections from landraces.
- Pedigree selection: Tracking ancestry and selecting superior lines through multiple segregating generations improved breeding efficiency. Research shows that pedigree breeding typically achieves genetic gains of 0.5-1.2% annually for yield in self-pollinating crops.
- Backcross breeding: Incorporating specific traits while recovering the desirable genetic background of adapted varieties enabled targeted improvement. Studies demonstrate that modern backcross breeding using molecular markers can transfer target genes while recovering 97-99% of the recurrent parent genome in 2-3 generations compared to 6-8 generations with conventional approaches.
- Heterosis Exploitation and Hybrid Development
Capturing hybrid vigor revolutionized production in many crops:- Hybridization systems: Development of practical methods to produce hybrid seed enabled commercial exploitation of heterosis. Historical analyses show that the introduction of hybrid maize increased US yields by approximately 15% immediately and enabled sustained annual gains of 1-2% thereafter.
- Heterotic patterns: Identifying complementary genetic groups that produce superior hybrids when crossed enhanced breeding efficiency. Research demonstrates that well-established heterotic patterns in maize increase hybrid performance by 10-15% compared to crosses between unassigned lines.
- Cytoplasmic male sterility and fertility restoration: Biological systems for hybrid seed production expanded hybridization to new crops. Studies show that hybrid rice yields 15-30% more than conventional varieties, contributing to food security for millions of people since its commercial introduction in the 1970s.
- Mutation Breeding and Polyploidy
Artificially inducing genetic variation expanded breeding possibilities:- Mutation induction: Using radiation or chemical mutagens to create novel variation provided new traits. The FAO/IAEA Mutant Variety Database documents over 3,400 officially released mutant varieties across 170 plant species, including disease-resistant barley, semi-dwarf rice, and high-oleic sunflower.
- Polyploidy manipulation: Chromosome doubling created new species and improved existing ones. Research shows that induced polyploidy has contributed to 20-25% of all crop varieties, particularly in ornamentals, fruits, and some grains, with polyploids typically showing 15-40% larger organs and higher stress tolerance than their diploid progenitors.
- Haploid technology: Producing doubled haploid plants accelerated breeding by achieving homozygosity in a single generation. Studies demonstrate that doubled haploid technology reduces variety development time by 3-4 years in crops like maize, barley, and wheat while allowing evaluation of twice as many breeding populations with equivalent resources.
- Molecular Breeding Approaches
DNA-based methods dramatically enhanced selection precision and efficiency:- Marker-assisted selection: Using DNA markers linked to desired traits improved selection accuracy for specific genes. Meta-analyses indicate that marker-assisted selection increases breeding efficiency by 20-60% for simply inherited traits compared to phenotypic selection alone.
- Quantitative trait locus (QTL) mapping: Identifying genomic regions controlling complex traits enabled more effective improvement. Research demonstrates that major QTLs typically explain 10-30% of phenotypic variation for complex traits, with successful deployment improving target traits by 5-30% depending on genetic architecture.
- Genomic selection: Predicting performance based on genome-wide marker effects revolutionized breeding for complex traits. Studies show that genomic selection has increased annual genetic gain rates by 20-100% in various crops by reducing cycle time and increasing selection accuracy, with particularly strong impacts in perennial crops where conventional breeding cycles exceed 5-10 years.
- Genetic Engineering and Genome Editing
Precise modification of plant genomes enabled targeted trait improvement:- Transgenesis: Introducing genes from any organism expanded the range of achievable traits. Meta-analyses of field trials show that insect-resistant transgenic crops increase yields by 10-25% in high pest pressure environments while reducing insecticide applications by 40-80%.
- Cisgenic and intragenic approaches: Transferring genes between crossable plants or rearranging a plant’s own genetic elements provided intermediate options. Research demonstrates that cisgenic apple varieties with durable disease resistance from wild apple genes reduce fungicide applications by 60-90% while maintaining fruit quality identical to conventional varieties.
- CRISPR-Cas genome editing: Making precise changes to existing genes enables subtle modifications with potentially significant effects. Studies show that CRISPR editing has successfully improved traits like disease resistance, drought tolerance, nutritional quality, and yield components across major crops, with edited rice varieties showing 25-30% higher yield under drought conditions and edited tomatoes containing 5-6 times more GABA (gamma-aminobutyric acid) than conventional varieties.
Applications and Impacts Across Key Agricultural Objectives
Plant breeding innovations have delivered substantial improvements across multiple dimensions of agricultural performance:
- Yield Enhancement and Food Security
Breeding has significantly increased food production capacity:- Genetic yield gains: Long-term studies document breeding-driven yield increases of 0.8-1.2% annually in wheat and rice, and 1.5-2.0% in maize over the past 50 years. These genetic improvements account for approximately 50-60% of overall yield gains, with the remainder attributed to improved agronomy.
- Harvest index optimization: Changing plant architecture to allocate more resources to harvestable components has enhanced productivity. Research shows that modern wheat varieties allocate 45-55% of biomass to grain compared to 25-35% in pre-Green Revolution varieties, directly contributing to 30-40% higher yields.
- Heterosis exploitation: Expanding hybrid breeding to more crops continues to unlock yield potential. Studies demonstrate that hybrid cotton yields 20-30% more than conventional varieties, while hybrid rice has increased productivity by 15-25% in areas where it has been widely adopted.
- Yield stability: Developing varieties that perform well across varied environments enhances food security. Research shows that modern varieties typically show 15-30% less yield variation across environments than older varieties while maintaining higher average performance.
- Stress Resilience and Climate Adaptation
Breeding has enhanced crop ability to withstand challenging conditions:- Disease resistance: Incorporating genetic resistance to major pathogens protects production. Meta-analyses indicate that resistant varieties reduce yield losses by 20-40% under disease pressure while decreasing fungicide use by 30-70%.
- Drought tolerance: Developing varieties that maintain performance under water limitation addresses a major constraint. Field trials demonstrate that drought-tolerant maize varieties yield 15-30% more under severe water stress and 5-10% more under moderate stress compared to conventional varieties.
- Heat tolerance: Improving crops’ ability to withstand high temperatures becomes increasingly critical. Research shows that heat-tolerant wheat varieties maintain 20-40% higher grain set during heat stress events exceeding 35°C during flowering.
- Salinity tolerance: Enabling production on salt-affected soils expands agricultural possibilities. Studies demonstrate that salt-tolerant rice varieties yield 30-50% more than conventional varieties when irrigation water contains 3-6 dS/m salt concentrations, expanding production potential to millions of hectares previously considered marginal.
- Nutritional Quality Enhancement
Breeding has improved the nutritional value of major food crops:- Biofortification: Increasing micronutrient content addresses hidden hunger. Clinical trials show that iron-biofortified beans and pearl millet can reduce iron deficiency in women and children by 30-40% within 4-6 months of regular consumption, while provitamin A-biofortified maize and cassava significantly increase vitamin A status in vulnerable populations.
- Protein improvement: Enhancing protein content and quality upgrades nutritional value. Research demonstrates that quality protein maize (QPM) contains 70-100% more lysine and tryptophan than conventional maize, improving protein utilization efficiency by 30-40% in human diets.
- Healthy oil profiles: Modifying oil composition creates healthier products. Studies show that high-oleic sunflower and soybean oils containing 75-90% oleic acid (compared to 20-30% in conventional varieties) exhibit 10-15 times greater oxidative stability while reducing LDL cholesterol when substituted for conventional oils in human diets.
- Antinutrient reduction: Decreasing compounds that limit nutrient absorption improves nutritional outcomes. Research demonstrates that low-phytate maize and beans increase zinc and iron bioavailability by 30-50%, potentially addressing key micronutrient deficiencies in plant-based diets.
- Resource-Use Efficiency
Breeding has enhanced crops’ ability to produce more with fewer inputs:- Nitrogen use efficiency: Developing varieties that yield more per unit of nitrogen applied reduces environmental impacts. Field trials show that modern maize hybrids produce 35-65% more grain per kg of nitrogen than varieties from the 1970s, with approximately half of this improvement coming from breeding and half from improved management.
- Phosphorus acquisition: Enhancing crops’ ability to access soil phosphorus improves performance in low-input systems. Research demonstrates that bean varieties with enhanced mycorrhizal associations and root architectural traits yield 20-40% more under low phosphorus conditions compared to conventional varieties.
- Water productivity: Breeding for traits that enhance water-use efficiency addresses scarcity challenges. Studies show that modern wheat varieties produce 20-30% more grain per unit of water compared to older varieties through improved harvest index and physiological adjustments.
- Weed competitiveness: Developing crops that better suppress weeds reduces herbicide dependence. Field trials demonstrate that competitive rice varieties can reduce weed biomass by 25-40% compared to non-competitive varieties, potentially reducing herbicide requirements by 15-30%.
- Postharvest Traits and Value Addition
Breeding has improved harvestability, storability, and processing quality:- Lodging resistance: Strengthening stems prevents crop collapse before harvest. Studies show that lodging-resistant cereals reduce harvest losses by 10-25% under adverse conditions while enabling higher nitrogen application rates for increased yields.
- Storage stability: Extending the shelf life of harvested products reduces losses. Research demonstrates that improved bean varieties with bruchid resistance reduce storage losses by 60-80% over 6-month storage periods without chemical treatments.
- Processing quality: Enhancing traits valued by processors increases product value. Studies show that malting barley varieties with optimized enzyme profiles improve brewing efficiency by 5-10%, while high-amylose wheat increases fiber content in baked goods by 4-6 times compared to conventional wheat products.
- Consumer traits: Breeding for flavor, texture, and convenience adds value throughout supply chains. Research indicates that improved sensory traits can increase consumer willingness to pay by 15-40% for fruits and vegetables and 5-15% for staple crops, creating value that can be shared across the value chain.
Challenges and Considerations in Plant Breeding Innovation
Despite impressive achievements, plant breeding faces several important challenges:
- Genetic Diversity Conservation and Access
Maintaining and utilizing crop genetic diversity presents ongoing challenges:- Genetic erosion concerns: Modern agriculture has reduced cultivated diversity in many regions. Studies estimate that 75% of crop genetic diversity was lost during the 20th century as farmers replaced diverse landraces with fewer improved varieties, though seed bank collections have preserved much of this diversity ex situ.
- International policy frameworks: The International Treaty on Plant Genetic Resources for Food and Agriculture and Convention on Biological Diversity govern germplasm exchange. Research shows that countries with facilitated access to diverse germplasm develop varieties with 15-30% higher performance under stress conditions compared to programs relying primarily on domestic materials.
- Digital sequence information: Debates about ownership and access to genetic sequence data impact breeding innovation. Studies indicate that open access to genomic resources accelerates breeding progress by 30-50% compared to restricted systems, particularly for minor crops and programs in developing countries.
- Participatory approaches: Involving farmers in breeding enhances relevance and adoption. Research demonstrates that participatory varietal selection increases adoption rates by 20-40% compared to conventional release approaches, with particularly strong effects in heterogeneous, stress-prone environments.
- Intellectual Property and Commercialization
Balancing innovation incentives with access concerns creates tension:- Plant variety protection systems: Legal frameworks providing exclusive rights to breeders operate differently across jurisdictions. Studies show that countries implementing effective PVP systems typically see private breeding investments increase by 40-120% within 10 years, though impacts vary greatly by crop and market context.
- Patent protection: Utility patents on plants, genes, and technologies create stronger exclusivity. Research indicates that highly restrictive IP environments can reduce innovation by smaller breeding programs by 30-50% while concentrating control in fewer companies.
- Farmers’ rights: Recognizing farmers’ contributions to conservation and development remains challenging. Case studies show that successful farmers’ rights implementation increases their participation in seed systems by 15-40% and improves conservation of local genetic resources.
- Open source breeding: Developing alternative innovation models that ensure ongoing access. Early results from open-source breeding initiatives show comparable innovation rates to proprietary systems for some crops while maintaining 100% access to developed varieties for further breeding.
- Regulatory Frameworks for New Breeding Technologies
Oversight of breeding innovations varies greatly:- Regulatory consistency: Different countries regulate similar technologies in fundamentally different ways. Analyses show that regulatory disparities increase commercialization costs by 50-300% and delay variety deployment by 3-5 years in restrictive jurisdictions compared to science-based approaches.
- Process vs. product approaches: Regulations focusing on the breeding process rather than product characteristics create inconsistencies. Research indicates that process-based regulation increases development costs by 10-50 million USD for transgenic crops compared to conventional varieties, while product-based approaches focus regulatory resources on actual risk factors.
- SDN-1, SDN-2, and SDN-3 distinctions: Different categories of genome editing receive varied regulatory treatment. Studies show that genome edits without foreign DNA integration (SDN-1) pose no greater risk than conventional breeding mutations, while creating regulatory distinctions increases R&D costs by 5-15 million USD per product.
- International harmonization efforts: Attempts to align regulatory approaches face scientific and political challenges. Economic analyses demonstrate that global regulatory harmonization could reduce development costs by 40-60% and accelerate technology adoption in food-insecure regions by 3-8 years.
- Technical and Biological Limitations
Some biological constraints continue to challenge breeding progress:- Linkage drag: Difficulty separating desired genes from linked deleterious alleles slows improvement. Research shows that introgressing traits from wild relatives typically introduces 5-50 Mb of linked sequence that can reduce yield by 3-15% unless broken through extensive recombination and selection.
- Negative genetic correlations: Unfavorable relationships between desirable traits complicate simultaneous improvement. Studies demonstrate negative correlations of -0.3 to -0.7 between grain yield and protein content in cereals, requiring specialized breeding approaches to make progress in both traits simultaneously.
- Domestication bottlenecks: Limited genetic diversity for some traits restricts improvement potential. Research indicates that severe domestication bottlenecks in crops like tomato and cotton reduced genetic diversity by 50-80% compared to wild progenitors, limiting variation for stress resilience traits.
- Genetic complexity: Many important traits are controlled by numerous genes with small effects. Quantitative genetic studies show that 40-60% of yield variation typically comes from hundreds of small-effect loci, each contributing less than 1% to phenotypic variance, making improvement more difficult than for simpler traits.
- Socioeconomic and Adoption Considerations
Developing improved varieties does not guarantee their uptake and impact:- Seed systems development: Functioning seed production and distribution systems are necessary for variety adoption. Studies show that inadequate seed systems limit improved variety adoption to 10-25% in many developing regions despite demonstrated yield advantages of 20-50%.
- Fit to farming systems: Varieties must integrate with existing agricultural practices and constraints. Research demonstrates that varieties developed without considering farm-level realities typically achieve only 30-60% of their yield potential in farmers’ fields, compared to 60-80% for varieties bred with farming system compatibility in mind.
- Gender-responsive breeding: Considering gender-differentiated trait preferences enhances relevance to diverse users. Studies show that explicitly incorporating women farmers’ preferences increases variety adoption rates by 15-35% in crops where women play major roles in production and processing.
- Market linkages: Commercial opportunities influence variety adoption decisions. Research indicates that varieties with clear market demand and price premiums achieve 2-3 times higher adoption rates compared to varieties with yield advantages alone.
Case Studies of Breeding Innovation Impact
Examining specific breeding successes illustrates the principles and approaches that drive innovation:
- Drought-Tolerant Maize for Africa (DTMA)
This initiative developed improved varieties for rain-fed farming in sub-Saharan Africa:- Breeding approach: Combining conventional, marker-assisted, and participatory breeding accelerated progress. Teams identified key drought-tolerance genomic regions explaining 20-30% of yield stability under stress and incorporated them into locally adapted germplasm.
- Performance results: Field trials demonstrated yield advantages of 20-35% under severe drought and 5-10% under moderate stress compared to commercial checks, with no yield penalty under favorable conditions.
- Adoption and impact: By 2020, drought-tolerant varieties covered 5+ million hectares across 13 African countries. Impact studies show household food security improvements of 30-40% and poverty reduction of 5-10% among adopting farmers, with benefit-cost ratios exceeding 7:1 at program level.
- Enabling factors: Success resulted from long-term investment, partnership structure crossing public and private sectors, and attention to seed system development alongside breeding advances.
- Submergence-Tolerant Rice (Sub1)
This precision breeding case deployed flood tolerance to vulnerable regions:- Breeding innovation: Marker-assisted backcrossing incorporated the SUB1A gene from a traditional variety into modern high-yielding varieties. The process recovered 97-99% of recurrent parent genomes while transferring the critical tolerance gene.
- Performance impact: Varieties survive complete submergence for 10-18 days compared to 3-7 days for conventional varieties. Field studies demonstrate 40-80% higher yields following submergence events with no sacrifice in normal-condition productivity.
- Adoption scale: Sub1 varieties have been adopted on 8+ million hectares across South and Southeast Asia. Economic analyses show additional rice production valued at $1.3+ billion between 2014-2020, with benefits flowing primarily to smallholder farmers in flood-prone regions.
- Success drivers: Targeted trait deployment addressing a specific, severe constraint with minimal disruption to other variety characteristics facilitated rapid acceptance by farmers familiar with the recurrent parent varieties.
- Disease-Resistant Bean Varieties in East Africa
This program addressed multiple constraints affecting a key food security crop:- Breeding strategy: Pyramiding multiple resistance genes against bean common mosaic virus, anthracnose, angular leaf spot, and root rots created durable protection. Breeders combined 4-6 resistance genes in market-preferred backgrounds.
- Yield impact: Resistant varieties deliver 50-100% higher yields under severe disease pressure and 20-30% higher yields under moderate pressure compared to local varieties, translating to 400-800 kg/ha additional production.
- Economic returns: Cost-benefit analyses show returns of $2-3.7 for each dollar invested in breeding and seed systems development. Household studies demonstrate 15-25% higher bean consumption and 30-40% increased income from bean sales among adopting farmers.
- Scaling approach: Developing decentralized seed production through farmer associations and small enterprises increased seed access, achieving 60-75% adoption rates in target regions compared to 20-30% typical of centralized distribution systems.
- Genome-Edited High-GABA Tomatoes
This product exemplifies precision improvement of nutritional and quality traits:- Technical approach: CRISPR-Cas9 genome editing modified glutamate decarboxylase genes to prevent negative feedback inhibition, resulting in gamma-aminobutyric acid (GABA) accumulation.
- Nutritional impact: Edited tomatoes contain 5-6 times higher GABA levels than conventional varieties, potentially delivering calming, blood pressure-lowering, and other health benefits associated with this neurotransmitter.
- Regulatory pathway: In Japan, these tomatoes became the first CRISPR-edited food approved for commercial sale based on product-focused regulation that recognized the edit’s similarity to natural mutations.
- Consumer engagement: Transparent communication about the breeding technique and potential benefits led to consumer acceptance rates of 70-80% among Japanese consumers compared to 30-40% typical acceptance rates for transgenic products.
- Hybrid Rice Development and Deployment in China
This program transformed production of a staple crop:- Breeding innovation: Developing practical three-line and two-line hybrid systems overcame the self-pollinating nature of rice. Researchers identified wild-derived cytoplasmic male sterility systems and complementary fertility restorers that enabled commercial hybrid seed production.
- Yield advantage: Hybrids show 15-30% yield increases over conventional varieties through heterosis expression. National statistics demonstrate that hybrid rice contributed approximately 65% of Chinese rice production increases since the 1980s.
- Adoption scale: Hybrid rice now occupies over 50% of China’s rice area (16+ million hectares) and has spread to 40+ countries. Economic analyses credit hybrid rice with feeding an additional 70 million people annually in China alone.
- Continuous improvement: Second-generation hybrids using improved parental lines and better breeding methods show 10-15% yield gains over first-generation hybrids, demonstrating ongoing innovation potential.
Future Directions and Emerging Approaches
Several important trends promise to further enhance plant breeding capabilities:
- Speed Breeding and Accelerated Crop Improvement
Reducing breeding cycle time dramatically increases genetic gain rates:- Optimized growth environments: Controlled conditions with extended photoperiods accelerate generation advancement. Studies show that speed breeding protocols reduce generation time by 50-65% in wheat, barley, and chickpea while maintaining normal plant development and seed quality.
- Gene editing for early flowering: Modifying flowering-time genes enables rapid cycling. Research demonstrates that editing FT (FLOWERING LOCUS T) orthologs can reduce generation time by 60-80% in fruit trees and other perennials, potentially transforming breeding in these long-cycle crops.
- Machine learning prediction: Forecasting performance earlier in the life cycle reduces selection cycle time. Studies show that hyperspectral imaging combined with machine learning can predict yield performance at seedling stage with 70-85% accuracy compared to full-season evaluation.
- Economic impact: Modeling indicates that halving breeding cycle time while maintaining selection accuracy increases the net present value of breeding programs by 35-70% due to earlier variety deployment and faster response to emerging challenges.
- Predictive Breeding and Big Data Integration
Leveraging diverse data sources enhances selection decisions:- Multi-omics integration: Combining genomics, proteomics, metabolomics, and phenomics data improves prediction accuracy. Research shows that integrating transcriptomic data with genomic information increases prediction accuracy by 10-25% for complex traits compared to genomic data alone.
- Environmental prediction: Incorporating environmental data into breeding models enhances adaptation targeting. Studies demonstrate that models including high-resolution environmental covariates improve yield prediction accuracy by 15-30% across diverse locations compared to standard genomic prediction.
- Historical crop performance data: Leveraging decades of variety trial data enhances prediction models. Analyses show that incorporating historical performance trends improves breeding value estimates by 10-20%, particularly for environmental resilience traits.
- Artificial intelligence applications: Machine learning algorithms optimize complex breeding decisions. Simulations indicate that AI-optimized mating designs can increase genetic gain by 15-35% compared to conventional approaches by better exploiting complementarity and managing genetic diversity.
- Biological Insight-Driven Breeding
Deeper understanding of plant biology enables more targeted improvement:- Pathway engineering: Modifying complete metabolic or developmental pathways rather than single genes enhances complex traits. Research shows that engineering C4 photosynthetic pathways into C3 crops could theoretically increase productivity by 20-50%, with incremental progress demonstrating technical feasibility.
- Microbiome optimization: Selecting for beneficial plant-microbe interactions improves performance. Studies demonstrate that breeding for enhanced mycorrhizal responsiveness increases phosphorus efficiency by 15-40% in low-input systems, while recruiting beneficial rhizobacteria can improve stress tolerance by 20-30%.
- Epigenetic breeding: Exploiting non-genetic inheritance mechanisms expands improvement options. Research shows that epigenetic variation contributes 5-15% of heritable phenotypic variation in some crops, offering additional breeding levers beyond sequence modification.
- Structural variation utilization: Exploiting large-scale genomic rearrangements provides new variation. Studies reveal that structural variants contribute 20-40% of functional genetic diversity in many crops, with copy number variations explaining significant portions of trait variation not captured by single nucleotide polymorphisms.
- Democratization of Breeding Technologies
Making advanced tools accessible to diverse breeding programs enhances global impact:- Low-cost genotyping platforms: Affordable DNA analysis enables smaller programs to implement molecular breeding. Economic analyses show that genotyping costs below $5-10 per sample make genomic selection cost-effective even for crops with limited breeding investment.
- Open-source breeding informatics: Publicly available data management and analysis tools reduce entry barriers. Case studies demonstrate that open-source breeding management systems increase operational efficiency by 20-40% and enable advanced methodologies in previously resource-limited programs.
- Breeding API development: Standardized data interfaces facilitate tool integration and collaboration. Early implementations show 30-50% reductions in data processing time and 20-30% improvements in cross-institutional collaboration efficiency.
- Capacity development models: Training and support networks accelerate technology adoption. Evaluations indicate that comprehensive capacity building combining technical training, equipment access, and ongoing mentorship increases advanced methodology implementation success rates by 60-80% compared to training alone.
- Transdisciplinary Integration
Connecting breeding with other disciplines enhances relevance and impact:- Farmer participatory research: Including end-users throughout the breeding process improves adoption. Studies show that varieties developed with farmer input from initial stages achieve 30-50% higher adoption rates and 15-30% greater on-farm performance than those developed in isolation.
- Nutrition-sensitive breeding: Explicitly incorporating nutritional objectives alongside agronomic traits addresses multiple goals. Cost-effectiveness analyses indicate that biofortification through breeding is 4-30 times more cost-effective than supplementation or fortification for delivering micronutrients.
- Climate modeling integration: Using climate projections to set breeding targets enhances future adaptation. Research shows that breeding programs explicitly incorporating 10-30 year climate projections develop varieties with 15-25% better performance under emerging conditions than programs focused solely on current environments.
- Market systems alignment: Connecting breeding objectives with market opportunities creates value chain benefits. Case studies demonstrate that varieties developed with clear market specifications achieve 50-100% price premiums and 2-3 times faster adoption rates compared to varieties focused solely on production traits.
Conclusion
Plant breeding represents a remarkable fusion of science, art, and practice that has continuously evolved to address changing agricultural challenges. The progression from early farmer selection through systematic hybridization to today’s precision molecular approaches has delivered steady productivity increases while addressing emerging constraints. Modern breeding integrates diverse approaches—from conventional cross-hybridization to genome editing—within comprehensive improvement strategies tailored to specific crops, environments, and objectives.
The evidence demonstrates that plant breeding has been responsible for approximately 50-60% of realized yield gains across major crops, while also delivering critical improvements in stress resilience, resource-use efficiency, nutritional quality, and environmental adaptation. These achievements have been essential to food security gains despite growing populations and emerging challenges like climate change, evolving pest pressures, and resource limitations.
Looking forward, the integration of genomic tools, high-throughput phenotyping, environmental modeling, and participatory approaches promises to further accelerate genetic gain while addressing the complex challenges facing 21st-century agriculture. Realizing this potential requires thoughtful navigation of policy considerations around genetic resource access, intellectual property protection, regulatory frameworks, and technology democratization.
Successful plant breeding must ultimately deliver varieties that not only perform well experimentally but also meet the needs of farmers, consumers, processors, and ecosystems. This requires breeding programs that balance multiple objectives, engage diverse stakeholders, and consider the full context in which crops are grown and used. By embracing this comprehensive perspective while leveraging cutting-edge scientific tools, plant breeding can continue its essential role in creating more productive, resilient, nutritious, and sustainable agricultural systems capable of meeting humanity’s food and environmental needs.
References
- Hickey, L. T., Hafeez, A. N., Robinson, H., Jackson, S. A., Leal-Bertioli, S. C., Tester, M., Gao, C., Godwin, I. D., Hayes, B. J., & Wulff, B. B. (2019). Breeding crops to feed 10 billion. Nature Biotechnology, 37(7), 744-754.
- Varshney, R. K., Bohra, A., Yu, J., Graner, A., Zhang, Q., & Sorrells, M. E. (2021). Designing future crops: Genomics-assisted breeding comes of age. Trends in Plant Science, 26(6), 631-649.
- Crossa, J., Pérez-Rodríguez, P., Cuevas, J., Montesinos-López, O., Jarquín, D., de los Campos, G., Burgueño, J., González-Camacho, J. M., Pérez-Elizalde, S., Beyene, Y., Dreisigacker, S., Singh, R., Zhang, X., Gowda, M., Roorkiwal, M., Rutkoski, J., & Varshney, R. K. (2017). Genomic selection in plant breeding: Methods, models, and perspectives. Trends in Plant Science, 22(11), 961-975.
- Bailey-Serres, J., Parker, J. E., Ainsworth, E. A., Oldroyd, G. E., & Schroeder, J. I. (2019). Genetic strategies for improving crop yields. Nature, 575(7781), 109-118.
- Li, H., Rasheed, A., Hickey, L. T., & He, Z. (2018). Fast-forwarding genetic gain. Trends in Plant Science, 23(3), 184-186.
- Pixley, K. V., Falck-Zepeda, J. B., Giller, K. E., Glenna, L. L., Gould, F., Mallory-Smith, C. A., Stelly, D. M., & Stewart Jr, C. N. (2019). Genome editing, gene drives, and synthetic biology: Will they contribute to disease-resistant crops, and who will benefit? Annual Review of Phytopathology, 57, 165-188.
- Halewood, M., Chiurugwi, T., Sackville Hamilton, R., Kurtz, B., Marden, E., Welch, E., Michiels, F., Mozafari, J., Sabran, M., Patron, N., Kersey, P., Bastow, R., Dorius, S., Dias, S., McCouch, S., & Powell, W. (2018). Plant genetic resources for food and agriculture: Opportunities and challenges emerging from the science and information technology revolution. New Phytologist, 217(4), 1407-1419.
- Atlin, G. N., Cairns, J. E., & Das, B. (2017). Rapid breeding and varietal replacement are critical to adaptation of cropping systems in the developing world to climate change. Global Food Security, 12, 31-37.
- Zhang, Y., Pribil, M., Palmgren, M., & Gao, C. (2020). A CRISPR way for accelerating improvement of food crops. Nature Food, 1(4), 200-205.
- Bevan, M. W., Uauy, C., Wulff, B. B., Zhou, J., Krasileva, K., & Clark, M. D. (2017). Genomic innovation for crop improvement. Nature, 543(7645), 346-354.
- Ricroch, A., Clairand, P., & Harwood, W. (2017). Use of CRISPR systems in plant genome editing: Toward new opportunities in agriculture. Emerging Topics in Life Sciences, 1(2), 169-182.
- Ashraf, M., & Foolad, M. R. (2013). Crop breeding for salt tolerance in the era of molecular markers and marker-assisted selection. Plant Breeding, 132(1), 10-20.
- Bohra, A., Chand Jha, U., Godwin, I. D., & Varshney, R. K. (2020). Genomic interventions for sustainable agriculture. Plant Biotechnology Journal, 18(12), 2388-2405.
- Khoury, C. K., Bjorkman, A. D., Dempewolf, H., Ramirez-Villegas, J., Guarino, L., Jarvis, A., Rieseberg, L. H., & Struik, P. C. (2014). Increasing homogeneity in global food supplies and the implications for food security. Proceedings of the National Academy of Sciences, 111(11), 4001-4006.
- McCouch, S., Baute, G. J., Bradeen, J., Bramel, P., Bretting, P. K., Buckler, E., Burke, J. M., Charest, D., Cloutier, S., Cole, G., Dempewolf, H., Dingkuhn, M., Feuillet, C., Gepts, P., Grattapaglia, D., Guarino, L., Jackson, S., Knapp, S., Langridge, P., … & Zamir, D. (2013). Agriculture: Feeding the future. Nature, 499(7456), 23-24.
If you want to learn more about Plant Breeding, From Traditional Selection to Genome Editing for Agricultural Resilience, check out Agri AI : Smart Farming Advisor and feel free to ask any questions!
Leave a Reply